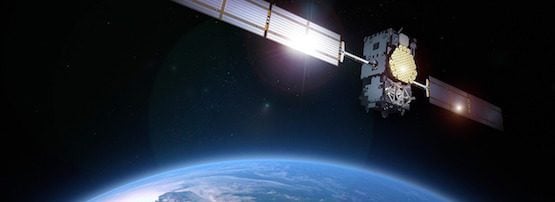
Far from being a vibrant environment with an array of competitive offerings, the activity of providing so-called “last mile” Internet access appears to have been reduced to an environment where, in many markets, a small number of access providers appear to operate in a manner that resembles a cosy cartel, strenuously resisting the imposition of harsher constraints of true competition.
Mobile access continues to operate at a distinct price premium and the choices for broadband wireline access is all too often limited to just one or two providers. Is there another option?
If we looked up into the sky, are there potential services that could alter this situation?
From my own experience, satellites played an important role establishing the first Internet transmission services that connected the Australian Academic and Research Network to the Internet’s infrastructure in the United States in the late 1980s, using an INTELSAT satellite circuit.
At that time, if you wanted an international data circuit, a satellite-based service was pretty much all that was available. We are talking about a pre-Web Internet, and much of the academic and research traffic was carried in asynchronous message-passing protocols, so the issues around latency and throughput were not overly relevant at that time. As long as the circuit had enough capacity to drain the queued message backlog eventually, then it met our basic requirements.
Of course, as with Maslow’s Hierarchy of Needs, once the basic requirement of connectivity was achieved, we moved on to wanting more – in this case, we wanted both higher capacity and lower latency over the circuit. Once the first of the South Pacific undersea fibre systems was completed, linking Australia to the United States in 1995, the Internet was an early customer.
The difference in the resulting service was truly stunning. Not only was there hundreds of megabits of capacity potentially available, but the round trip times for individual packet transactions across the Pacific shrunk from around two-thirds of a second to under a quarter of a second. It doesn’t sound like much, but when waiting for a web page to load, the subjective difference was truly incredible.
We were then hooked on speed, and we certainly never wanted to head back to satellite systems to cross the Pacific ever again. For us, and many others like us, the future of long haul intercontinental data transit was fibre. Whether it was under the seas or across the land, it was fibre that was our future.
But the satellite industry did not fade away. There is still a steady flow of new announcements of data services in the sky, and it seems as if space-based services are now positioning themselves to compete with terrestrial fibre systems on approximately equal terms.
There are a number of reasons for this. The first is an observation that there is a market niche that cannot be cost-effectively filled by terrestrial cable-based solutions, where satellite systems appear to excel.
Once the population density of a serviced area falls below approximately 10 people per square kilometre, then the per user cost of a terrestrial service starts to rise dramatically, while the per user cost of a satellite services remains roughly constant, making satellite an attractive proposition for low density rural and remote areas (study by Communications Research Centre, Canada, reported in ITU News, 2006).
While this is a result from a ten-year-old analysis, the underlying constraints that drive this outcome are still present. The cost of a fixed last mile service per users served rapidly escalates in rural and remote context where the population density is low. Satellite services require only the means to send and receive a signal from the spacecraft, and no other local infrastructure.
A second motivation concerns the continuing issues with the economics of last mile infrastructure in the Internet. The economics of installing a high-speed broadband infrastructure are proving challenging for the wireline folk, and many projects that intend to replace the copper-based telephone wiring with fibre systems continue to falter because of the high capital costs and anticipated low rates of return. In some markets, we’ve seen mobile operators attempt to fill this gap by deploying 4G systems, but they too have their issues with consistency of high-speed performance under load, as well as the relatively higher retail cost of the service in many markets.
Wouldn’t it be advantageous to a new market entrant in access services to solve these issues by using an approach that completely avoided the costs of this terrestrial infrastructure completely?
Space-based communications systems have been around for many decades now. In the US, the initial forays by AT&T, RCA and Hughes Aircraft in satellite communications in the early 1960s caused some alarm within the Kennedy administration over the incipient privatization of space. In response, the US created a public satellite corporation that was to be jointly owned by the public and by the major governmental contractors, called COMSAT.
There was a strong European reaction to this move, and in response, it was proposed to create a single world communications satellite system, 61% owned by COMSAT and the remainder owned by western European nations together with Canada, Japan and Australia. This corporate entity was called INTELSAT, and through the 1970s it launched a number of spacecraft into the geostationary orbital band to support a mix of international communications requirements, including television and telephone.
Various countries also launched spacecraft into slots in the same geostationary orbital band in the 1970s and 1980s, including Canada, France, Germany, Japan, the UK and India. This was similar in many ways to the very early years of computers: such high frontier projects were too big and way too important for mere private corporations to play in, and the space was dominated by national actors.
This model was broken in 1988 with the launch of PanAmSat, a project intended to break the de facto INTELSAT monopoly. The longer term consequences in the ensuing decade included the dismantling the COMSAT monopoly, and privatising both INTELSAT and its maritime equivalent, INMARSAT. Space is now the realm of both public and private sectors, and there now are a variety of services and further plans to populate the space above the Earth with communications satellites that compete not only with each other but directly with the terrestrial communications systems.
To look at this further, it’s probably useful to take a small diversion into physics.
Physical Constraints
The speed of propagation of electromagnetic radiation, including light, through a vacuum is approximately 300,000 km/sec.
The speed of light in a vacuum, or the physical sciences constant c, is probably the most researched constant in all of science. According to Einstein’s prediction about the speed of propagation of light within the general theory of relativity, the measured speed of light does not depend on the observer’s frame of reference; the speed of light in a vacuum is a universal constant.
Estimates of the value of c have been undergoing refinement since 1638, when Galileo’s estimate of “If not instantaneous, it is extraordinarily rapid” was published in Two New Sciences. The currently accepted value is 299,792.458 kilometres per second.
The speed of light in glass or fibre-optic cable is significantly slower, at approximately 194,865 km/sec.
The speed of propagation of electrical charge through a conductor is a related value; it, too, has been the subject of intense experimentation. Perhaps the most bizarre experiment was conducted in Paris, in April 1746, by Jean-Antoine Nollet. Using a snaking line of some 200 monks, connected by a mile-long iron wire, Nollet observed their reactions when he administered a powerful electric shock through the wire. The simultaneous screams of the monks demonstrated that, as far as Nollet could tell, voltage was transmitted through a conductor “instantaneously”.
Further scientific experimentation has managed to refine this estimate, and the current value of the speed of voltage propagation through a copper conductor is 224,844 km/sec, somewhat faster than the speed of light through fibre-optic cable.
The speed of light through the Earth’s atmosphere is a little slower, but when you guide this same photon through a piece of doped glass, as you find in a fibre optic cable, it is slowed down to some two-thirds of this original speed.
The speed of electrical propagation of a change along an electrical conductor has a number of variables, and the outcome is typically somewhere 0.75 and 0.9 times the speed of light in a vacuum. So, in very general terms, fibre is one of the slower transmission media, electrical propagation through a conductor is generally faster, and the fastest is direct propagation by light through a vacuum, or more generically, by electro-magnetic radiation propagation (or radio waves) through a vacuum.
Now we need to introduce a bit of conventional Newtonian physics. The thought experiment is relatively simple: if you fire a projectile with sufficient speed it will not fall back to earth. If you fire it at a speed greater than the Earth’s “escape velocity” (11.2 km/sec) it will head away from the Earth, never to return. If you reduce the speed slightly the projectile will be caught by the Earth’s gravity and try to fall back to earth. If you also incline the aiming trajectory, and if you fire the object sufficiently quickly, then instead of falling back to each, it will settle into an orbit around the Earth.
The orbital speed relative to the Earth is a function of the altitude of the object. At very high altitudes, such as the moon, the orbital period is slower than the Earth’s rotation, while at very low altitudes the orbital period is down to hours. That implies that there is a mid-spot where the orbital period is the same as the Earth’s rotation.
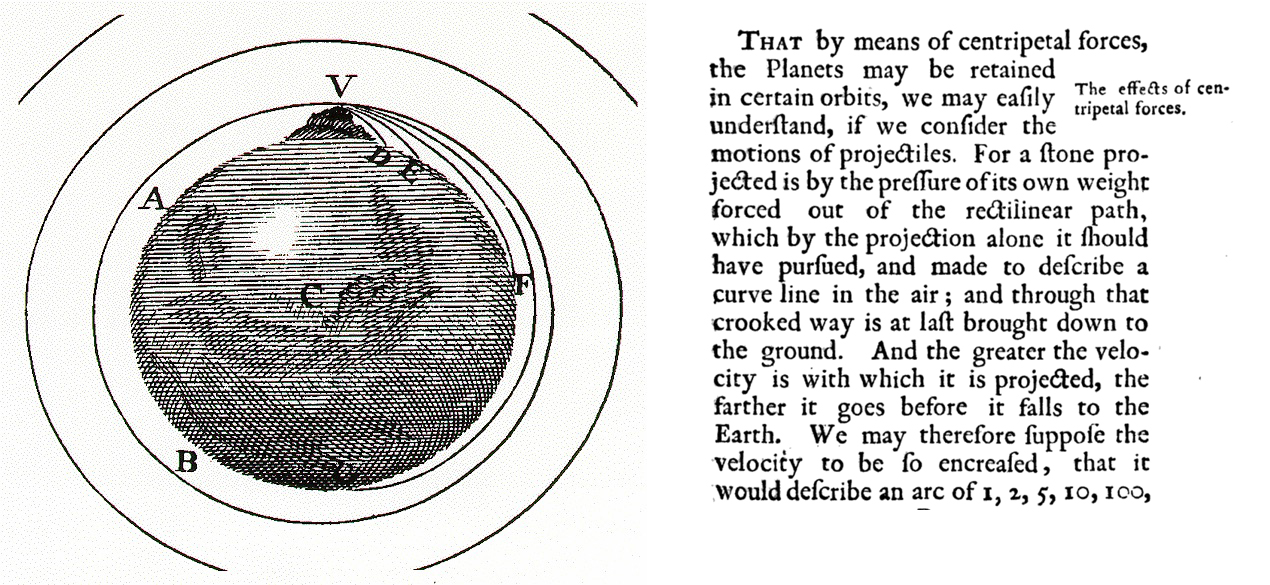
If you launch a satellite to an altitude of 37,568 km above the equator, orbiting in the same direction as the Earth on the equatorial plane, then from the Earth the spacecraft appears to sit in a stationary position when observed from the Earth’s surface. This means that earth-based satellite transmission and receiving equipment can be aligned towards a fixed position in the sky, rather than requiring tracking through constant realignment.
But 37,568 km is a long way up, and while the speed of propagation is now close to the speed of light, the distance from the Earth to the spacecraft and back is now some 72,000 to 80,000 km, depending on the position of the Earth stations relative to the spacecraft.
Even at the speed of light through space, it takes some time for a signal to travel from the Earth to the geostationary orbiting spacecraft and back. For example, for ground stations at latitudes of 45° on the same meridian as the satellite, the time taken for a signal to pass from Earth to the satellite and back again is approximately 260 ms.
An early use case for satellite communication was to support voice communications. But with a latency of approximately one-quarter of a second between the speaker and the listener, this introduced some further issues.
For voice, human perception studies noted that once the latency in communications passes beyond some 250 ms we tend to talk over each other, and need to revert to an explicit protocol to inform the other party we have stopped speaking.
For data transactions, these human factors should recede in importance. After all, the protocols that control an end-to-end data flow can be tolerant of these end-to-end delays and still ensure that the circuit can be used efficiently. But when we look at human use of network applications, we are normally looking at the time from initiating a transaction to the time of completion.
In the context of the Web we are talking about the time from clicking on a link to the page load. This time typically involves several preliminary transactions, including the resolution of domain names to IP addresses, a transport layer synchronisation handshake, and the negotiation of transport layer session encryption. Clearly, it’s not a single round trip time, but somewhere between four and eight such intervals. What would seem fast on a fibre-based route with a one-way delay of a couple of hundredths of a second may seem to be glacially slow over a route that entails a hop via a geostationary satellite with a one-way delay of a quarter of a second or more.
The perennial question for satellite-based communications is: how can we reduce this latency?
The speed of light is a constant, and the physical dimensions of the Earth are largely immutable, so if we want to reduce the time it takes for a signal to pass from Earth to the spacecraft and back, then one of the few remaining variables is to reduce the orbital radius of the spacecraft.
There is no clear boundary between the Earth’s atmosphere and space, as the density of the atmosphere gradually decreases as the altitude increases. A conventional definition used in international treaties is the so-called Kármán line, which is the altitude of 100 km above sea level. At that altitude, a vehicle would need to travel faster than orbital velocity to derive sufficient atmospheric lift to sustain its altitude. In other words, above this height an object is no longer flying using atmospheric lift, but orbiting.
Let’s look at these orbital zones in a bit more detail.
![[By Rrakanishu – Own work, GFDL]](https://blog.apnic.net/wp-content/uploads/2017/04/Orbitalaltitudes.jpeg)
Low Earth Orbit
An orbiting spacecraft needs to be positioned at least 160 km above the surface of the earth or it will encounter significant drag from the top of the earth’s atmosphere and its orbit will quickly decay, with an inevitable result. Above this altitude, it is viable to position orbiting spacecraft without needing to provide large quantities of continual propulsion (although some residual drag is experienced in orbital altitudes up to 500 km or so). For example, the International Space Station orbits at an altitude of 400 km, with an orbital period of some 90 minutes.
There are several advantages to an LEO orbit for communications satellites.
First, it requires less energy to place a spacecraft into an LEO orbit. Because the distance to the Earth is so much shorter, the spacecraft can use less powerful signal amplifiers, so it can be smaller and lighter, reducing launch costs and reducing the amount of propellant needed to manoeuvre the craft when in orbit.
From the Earth side, it is possible to use relatively small antennae to communicate with the spacecraft from the Earth’s surface. Communications with a spacecraft in an LEO orbit can be extremely fast. If you were directly under an LEO, the time to send a signal from the spacecraft can be as low as 3 ms.
However, to create a continuous service it is necessary to launch a constellation of such spacecraft, as each individual satellite in an LEO orbit will be travelling quickly across the sky relative to a fixed point on the Earth. The closer the orbit to the Earth, the faster each spacecraft will need to travel, and the greater the number of satellites needed to provide continuous service to any point on the Earth.
At the upper parts of this Orbital group, there is the Van Allen belt, a region that contains high concentrations of electrons in the range of hundreds of keV and energetic protons with energies exceeding 100 MeV, trapped by the Earth’s magnetic fields in the region. This has the potential to damage the solar panels and electronics carried on spacecraft, and ultimately to disable the unit.
Medium Earth Orbit
There is a family of orbital distances collectively referred to as “Medium Earth Orbits” or MEOs. This covers the range of altitudes from 2,000 km to the geostationary orbital altitude of 35,768 km. At the low end of this range, 2,000 km, the orbital period is around 2 hours, and the time to pass a signal up to the space craft and back is some 13ms if you are located directly under the spacecraft.
A single spacecraft orbiting in this orbital range would only be visible for some of the time: the spacecraft would appear to rise above the horizon, track across the sky and set below the opposite horizon.
Receiving signals from spacecraft orbiting at this altitude is possible with handheld devices, as illustrated by GPS positioning services, but sending to it may not be as simple as using a handheld device with a small antenna. Spacecraft in an MEO orbital band would normally need to be tracked if you are using some form of dish to communicate with it by sending directed radio signals to it.
For a continuous service at this altitude, you would still need a set of spacecraft, so that as one sets below the horizon, another spacecraft is already visible, but this set would be smaller than what is needed for an LEO constellation to provide continuous service.
The higher the altitude the longer the orbital period, which, in turn, reduces the number of spacecraft required to support a continuously available service. For example, the GPS positioning system uses a set of 31 spacecraft orbiting at some 20,200 km, Glonass, the Global Navigation System, uses 24 orbiting spacecraft at an altitude of 19,100 km and the Galileo positioning system is positioned in an MEO band at an altitude of 23,222 km. The transmission time to bounce a signal off the spacecraft at that altitude is around 140 ms.
This zone encompasses the inner and outer Van Allen belts. These belts protect the Earth’s atmosphere from being blown away by solar radiation (as appears to have happened to Mars once its inner core solidified). However, the not so good news for satellites is that orbiting in this belt is like wandering through a firing range – there is always the possibility the sensitive electronics are damaged by a strike from one of these energetic particles. The outer belt is less dense, but the particles can have significantly higher energy levels.
Beyond the Van Allen belts, spacecraft encounter far higher levels of risk of damage from cosmic rays and solar radiation. A region between the inner and outer Van Allen belts lies approximately 12,000 to 24,000 km in altitude, which has a lower incidence of such energetic particles. The belts fluctuate in size and shape due to changes in the levels of solar radiation, and the Earth itself acts as a shield. This means the belt is more compressed facing towards the sun and extends further out on the ‘dark” side of the Earth.
Geostationary Earth Orbit
As already noted, the orbital band at an altitude of 35,768 km has the unique property that the period of orbit is the same as the period of rotation of the Earth about its own axis. If the spacecraft is orbiting on the equatorial plane in the same direction as the Earth, then the spacecraft will appear to occupy a fixed position in the sky. This means that earth antennae can be mounted on a fixed pedestal, aimed at a constant position in the sky.
There are some 250 spacecraft positioned in geostationary orbital slots. Some of these satellites are separated from each other by as little as one-tenth of a degree longitude, which corresponds to an inter-satellite spacing of approximately 73 km. The major consideration for spacing of geostationary satellites is the beamwidth at-orbit of uplink transmitters, which are positioned 35,768 km away. This is primarily a factor of the size and stability of the uplink dish, as well as what frequencies the satellite’s transponders receive. Satellites with non-overlapping frequency allocations can be positioned much closer together.
There have been efforts by various countries located under the equator to claim the geostationary slots directly overhead as some part of their territorial domain, but these claims have not managed to gain any credence in the international community. The population of the geostationary orbital space, and the spectrum allocations used by these spacecraft are coordinated through the Space Services Division of the International Telecommunications Union (ITU), part of the ITU’s Radiocommunication Sector (ITU-R).
Radio Frequencies
As well as orbital altitude, there is the consideration of the radio frequency used to communicate with the spacecraft.
There are a number of frequency bands used to communicate with satellites:
Radio frequency | Type | Downlink frequency |
VHF | Very High Frequency | 30-300 MHz |
UHF | Ultra High Frequency | 0.3-1 GHz |
L Band | Long Wave Band | 1-2 Ghz |
S Band | Short Wave Band | 2-4 Ghz |
C Band | 4-8 Ghz | |
Ku Band | 10-18 Ghz | |
Ka Band | 26-49 GHz |
Specialised services have been allocated some of these frequencies, such as the use of S Band for deep space and research activities, of L band for GPS and meteorological observational spacecraft. Satellite services commonly use C Band and Ku Band, and, more recently, Ka Band.
Higher frequencies can take advantage of smaller high gain antennas. For example, a C Band antenna requires 16 times the surface area of a Ka band antenna to produce the same amount of gain. The higher frequency channels also encompass a broader frequency range, allowing for systems with higher bit rates. These factors would tend to favour the higher frequency bands.
However, these frequencies have different rain absorption properties. The amount of attenuation increases as the density of the rain increases and as the wavelength of the electromagnetic wave approaches the size of a typical raindrop. The wavelength is equal to the speed of light (3 x 108 m/s) divided by the frequency, while the average diameter of a raindrop is about 1.5 mm. At the downlink C band frequency of 4 GHz, the wavelength is 75 mm. Thus, the wavelength is 50 times the average diameter of a raindrop and the waves pass through the rain virtually without interaction.
In the Ku band, with downlink frequency of 12 GHz, the wavelength is 25 mm. Here the ratio of wavelength to raindrop diameter is still fairly large but is one-third the value at C band. Consequently, there is an interaction that can result in a noticeable absorption of energy. The received carrier power will decrease and the bit error rate of a digital signal will increase.
At the Ka band downlink frequency of 20 GHz, the wavelength is 15 mm. At this frequency, the wavelength is only 10 times the size of a raindrop. Therefore, there is a significant exchange of energy between the passing electromagnetic waves and the rain and a corresponding significant attenuation of the satellite signal. As the wavelength approaches 1.5 mm, the attenuation continues to increase and theoretically becomes maximum at a frequency of 200 GHz. What this implies is that to achieve the same parameters as a C band system, a KA band system would need to use a far larger antenna in order to overcome rain absorption.
The C band encompasses some 4 Ghz of spectrum, Ku band encompasses 8Ghz and Ka band encompasses 23Ghz. The major advantage of Ka band is the higher potential bandwidth per channel. The combination of low orbital planes, a large array of spot beams allows for very high capacity space-based systems.
Selected Current and Future Satellite Communications Systems
GEOs
Intelsat is one of the more venerable systems. It started in 1964 as an intergovernmental organisation intended to provide space-based services and provided voice trunk services to telcos as well as television distribution services. The organisation was privatized in 2001.
Intelsat operates a constellation of 54 spacecraft located in geostationary orbital slots, operating predominately in the C and Ku bands, with a small complement of Ka band transceivers. The model of operation is a simple “up-and-back” model, linking earth stations via a common satellite. There is no inter-satellite communications in this model.
In many ways, Intelsat services compete with long haul terrestrial trunk fibre systems, providing wholesale connectivity services between two earth stations. They cannot service the polar regions as there is no clear line of sight. They use targeted spot beams as a means of increasing the amount of spectrum reuse by the spacecraft.
Inmarsat has a similar inter-governmental background. The organization was established in 1979 at the behest of the International Maritime Organization (IMO), the United Nations’ maritime body.
The organisation was created to establish and operate a satellite communications network for the maritime community on the high seas. Inmarsat was privatized in 1999, and acquired Stratos in 2009. They operate 12, soon to be 13, satellites in geostationary orbital slots.
Unlike Intelsat, Inmarsat provides services directly to handset users, and provides both telephony and medium capacity data services using a 492 Kbps shared data channel.
The most recent spacecraft, the three, soon to be four, Inmarsat-5 satellites, operate Ka band services using 89 spot beams and six fully steerable beams. The use of the Ka band allows a download data rate of some 60 Mbps to a 60 cm earth dish. They also plan to use this service to support a Ka band service to downlink to airplanes.
They have a range of services that have evolved from the original satellite phone, and they now support a “Broadband Global Area Network”, an IP network that is accessible from all non-polar parts of the planet. They have services that include higher data rates, data bursts (such as used in remote sensing), and a message service similar in nature to SMS mobile messaging services. The Inmarsat terminal devices are not quite handheld, as they require a slightly larger antenna.
The relative advantages of this approach are that a small number of spacecraft are needed to provide global coverage, and, if you have a line of sight to a spacecraft, then the only other issue is that of rain fade, depending on the frequency being used.
The disadvantage is that these are large spacecraft, at high orbit, which means the launch costs are high, and the distance from the Earth generally requires larger antennae for a resilient service. The satellites only operate in up-and-down mode, and typically connect a remote terminal to a gateway station service from a single spacecraft.
There are numerous other spacecraft positioned in geostationary orbital slots, used for broadcasting, meteorological observation, communications, and military purposes. A list of spacecraft positioned in geostationary orbit can be found on Wikipedia.
MEOs
There are a number of systems in MEO orbits, including the L Band services of GPS, Galileo, Compass, and Glonass.
A recently announced entrant in the MEO orbital space is the Laser Light Network. This has not been launched yet, but is intended to operate in a manner similar to that of Iridium, where each spacecraft is capable of communicating both with earth systems and with each other by laser signalling.
The network is to be based on eight to 12 spacecraft in an MEO orbit, and each spacecraft will be equipped with 48 200-Gpbs inter-spacecraft links, and 72 100-Gbps steerable up/down service links to earth. As is evident from this quick summary, it is yet to launch a service.
One recent MEO service that has launched is O3B. The name “O3B” was derived from “the other 3 billion”, referring to the population not serviced by conventional terrestrial broadband infrastructure, and a number Internet service provider networks in the Pacific appear to have signed up to O3B services.
O3B uses a constellation of 12 satellites in an MEO orbital slot in the equatorial plane at an altitude of 8,068 km, with a further 8 satellites to be launched in the next couple of years.
O3B uses Ka band communications and operates in a strict “up-down” mode with no inter-satellite handover. Each satellite is equipped with 12 fully steerable Ka band antennas (two beams for gateways, 10 beams for remotes) that use a total of 4.3 GHz of spectrum. Each beam supports 800 Mbps, in each direction, supporting a total of 16 Gbs of cross traffic on each of the spacecraft. Each beam’s footprint measures 700 km in diameter.
Being lower than geostationary orbits by a factor of four reduces the signal propagation latency by a similar factor. Earth gateway facilities require two tracking dishes, so that at any time one is locked on to the overhead craft, with the other is seeking the next spacecraft as it rises above the horizon.
LEOs
One of the earlier LEO-based services was the Iridium constellation, developed by Motorola in the 1990s. This service used a constellation of 77 satellites (pared down to 66 in the design phase), launched into a polar orbit at an orbital height of 780 km.
Each orbital path consists of 11 spacecraft evenly spaced along the path. At the equator, each spacecraft is visible for just seven minutes. Each spacecraft is equipped with a number of spot beams and communication is routed between spot beams every 50 seconds. Iridium operates using L Band at 1616-1625Mhz. This is predominately a voice service with some small capacity data circuits also available.
Iridium spacecraft can communicate with neighbouring spacecraft within the Iridium constellation using Ka Band communications. This is perhaps the most innovative part of the entire system. Other constellations, such as Globalstar, are in effect orbiting mirrors, where each individual satellite takes an upcoming signal and reflects it back to an earth station. While it’s possible to create a configuration of orbiting spacecraft that covers the entire earth’s surface, useful coverage is limited by the number and location of ground stations in this model.
Iridium overcame this by its ability to pass the signal to another spacecraft. At its most extreme case, Iridium only needs one ground station, and all downward signals would be passed to the spacecraft that was in “view” from the ground station at any time. More ground stations improve the overall capacity of the system by augmenting the bandwidth of the space-to-ground links, and improve the service by reducing the terrestrial path from the nearest ground stations to the intended signal terminal point, but do not alter the original property of comprehensive global service.
Iridium has an interesting genesis where following the initial launch in November 1998 it entered Chapter 11 bankruptcy proceedings nine months later in August 1999. The original 6 billion USD investment was purchased by a private investment consortium for some 35 million USD in 2000. Since then, Iridium has survived and even prospered, so much so that it is now in the process of launching 70 Iridium NEXT satellites to replace the existing constellation and augment its services with more data capabilities.
Globalstar has had a similar troubled initial path to Iridium. It was conceived by the aerospace corporation Loral and the communications corporation, Qualcomm. Globalstar launched 48 satellites into an LEO orbital plan by February 2000 and sought Chapter 11 Bankruptcy protection in February 2002. The system offers mobile telephony services and messaging. The spacecraft are inclined off the equatorial plane by 52 degrees, and orbit at an altitude of 1,400 km. The system operates without spacecraft-to-spacecraft communications, so all communications operate in an up-down mode from the same spacecraft. This, in turn, implies that serviced areas required ground “gateways” to interface satellite calls into the terrestrial telephone system, and this has limited the ability of Globalstar to provide a truly global service. A second generation of this constellation was launched between 2011 and 2013.
Orbcomm, a satellite constellation also in LEO orbit was intended to primarily perform asset tracking functions. From 1997 to 1999, Orbcom launched 35 satellites, and then in September 2000 it also filed for Chapter 11 bankruptcy protection. The first generation of satellites were replaced by 18 second generation satellites between 2010 and 2014, and Orbcom have consolidated their business focus on M2M small burst communications, primarily involved with asset tracking.
On the starting block is OneWeb, a startup that plans to launch a constellation of 648 150 kg “micro-satellites” into the LEO orbital band, starting late 2017. In February 2017, OneWeb announced that it had sold most of the capacity of its initial 648 satellites, and was considering nearly quadrupling the size of the satellite constellation by adding 1,972 additional satellites to the constellation.
At the end of February 2017, they announced a merger with Intelsat, combining Intelsat’s geostationary orbital fleet with a larger set of LEO systems. The initial set of 648 satellites will operate on 20 orbital planes, and use Ku band communications. They have announced a capacity of 6 Gbps per satellite, and an aggregate network capacity of some 4.2 Tbps. They appear to be focussing on a form of ubiquitous web access as their target market sector.
Also on the cards is LEOSAT, a constellation of up to 108 satellites, where each satellite is configured with 10 Ka Band steerable user antennas. Each satellite will be capable of providing customer terminals with between 50 Mbps and 1.6 Gbps of symmetrical data connectivity, and two steerable gateway antennas, capable of an aggregated throughput of up to 10 Gbps, and four optical inter-satellite links. The intended scope of operation for LEOSAT is secure high-speed, low-latency, long haul point-to-point virtual circuits for the enterprise market.
SPACEX have also announced a set of intentions, and evidently are planning the deployment of some 4,000 cross-linked communications satellites in an LEO orbital band of 1,100 km, intended to support a global broadband Internet service, using Ku and Ka bands. While details are scant at this time, it appears to be the most ambitious of these LEO projects and perhaps one that could pose the largest threat to the current terrestrial systems that provide data access services to consumers.
Where now?
For many years, the last mile of connectivity has proved to be a formidable challenge for service providers. The economics of burying cables in the ground for decades has an associated investment model that often calls for public support or other forms of public subsidy in order to compete with other capital investment opportunities. At the same time, the exclusive-use spectrum model has, with few exceptions, priced cellular radio access services at a considerable premium, so that instead of a competitive market consumers appear to be faced with a cartel-like situation where “good, fast and cheap” is yet again proving to be an unachievable triumvirate in this closed situation.
From the euphoria of the late 1990s and the subsequent sobering bankruptcies in the early 2000s, the satellite industry appears to be enjoying a new wave of investor interest. It does seem possible to launch a constellation of satellites into relatively low earth orbits that allow communications to almost anyone on Earth using access equipment that is little different to what is already used for the cellular services. The most exciting projects, which combine a large set of satellites, high capacity, inter-satellite switching and LEO orbital planes, are still in the phase of raising capital and refining their designs, but they look like they will be viable.
No doubt, the local terrestrial incumbents will try to protect their incumbency with bureaucratic barriers, but the enticing prospect remains that it appears to be possible these days to launch services into orbit that can offer viable Internet services to literally everybody on the planet. And they appear to be able to do so at potential price points that appear to be closer to “widely affordable” as distinct from “first world luxury” pricing.
As always, these developments in communications technology continue to question our assumptions, challenge our conventions, and they offer us dazzlingly different opportunities. The future of the Internet may not end up being up there in the sky, but the competitive challenge these orbiting systems can pose to the terrestrial services will probably drive them both to achieve new standards of service, speed, and price, as long as they are given the opportunity to openly compete in the first place.
Acknowledgement
At the NZNOG 2017 meeting earlier this year, held in Tauranga, Jon Brewer presented his take on the satellite picture in a presentation “The Future is Up in the Sky”. Jon’s great presentation stimulated me to read more on this subject, and to write this summary of what I managed to find out. Thanks Jon.
The views expressed by the authors of this blog are their own and do not necessarily reflect the views of APNIC. Please note a Code of Conduct applies to this blog.
Great article Geoff!
A couple of comments:
– Until relatively recently, satellite Internet was synonymous with geostationary satellites. While this is changing, it’s worth pointing out that there is inequality in the sky, too, when it comes to provisioning Internet to areas with low population density. Take two examples: An island in the middle of the Pacific, and a remote village in northern Canada. Now take up the position of a geostationary satellite (hint: for visualisation, start Google Earth and zoom out to an eye altitude at geostationary orbit). Above the Pacific, most of your visible area is water, and the number of customers you are looking at is very small: Population density is < 10 / sq km but for the most part it's 0. Change your longitude to the Americas, and you see plenty of areas where population density is < 10 / sq km but larger than 0. This means you can recoup some of the cost of provisioning satellite Internet to your Canadian village by spreading the cost across many other potential customers in the Americas. In the Pacific, that's not so easy. As a result, much of the R&D in satellite systems has tended to focus on how to get service to users in Europe, Africa, and the Americas.
– One of the reasons why satellite Internet (especially geostationary) is not as nice as fibre is that the Transmission Control Protocol (TCP) doesn't really work well via comparatively narrow-band links with long latency, especially if these are shared between multiple TCP senders. This leads to effects such as long slow start phases or TCP queue oscillation, which leave links underused and deprive users of some of the capacity they have purchased.
The latter effect is understood reasonably well in the Internet engineering community, whose primary packet loss model is based on queue drops. However, it doesn't necessarily feature on the radar of engineers who design satellite equipment. Their packet loss model revolves around bit errors / symbol errors as a result of noise / fading / distortion etc. Since their primary markets can afford significant bandwidth, the needs of small markets that can afford narrow-band service only don't feature that prominently.
MEO and LEO concepts are promising for the Pacific: For one, they promise better service with lower latency and higher bandwidth at lower cost. They are also more robust in the face of cyclones, which are a fact of life in a lot of Pacific islands: Big dishes for GEO sats are inherently more at risk than small ones for MEO – and the size difference is substantial. However, where mechanical tracking of satellites is involved, there are more parts involved, some of whom move, and so commissioning and maintenance can be an issue if the community served is really remote. It's not uncommon for remote islands to go incommunicado for many months when their equipment breaks down – it takes that long to get a replacement and/or technician in on the next ship. Again, not normally an issue for northern Canada!
India to enter high-speed internet era with Isro’s new satellites
http://timesofindia.indiatimes.com/india/new-isro-satellite-launches-to-usher-in-high-speed-internet-era-in-india/articleshow/58769896.cms
This is an incredibly detailed write up of the state of satellite communications.
Fun fact: the first telecommunications satellite, Telstar 1, was in serivce less than 6 months before it was rendered inoperable by atmospheric nuclear weapons tests: https://en.wikipedia.org/wiki/Telstar#In_service